Almost immediately after its discovery in
1911, superconductivity and superconducting wires, with their
ability to carry direct current without loss, were proposed for
electricity transmission and distribution cable application.
However, the early superconductors were primarily elemental metals
whose superconducting properties disappeared for even moderate
currents and magnetic fields. Furthermore, the necessity to supply
large amounts of liquid helium for their operation was a major, if
not overwhelming, barrier. Not until the discovery of
“hard” superconducting alloys such as NiTi and Nb3Sn
capable of sustaining practical levels of current in the years
following World War II, the ability to manufacture long wire lengths
of these materials, and the increasing availability of efficient
helium liquefaction equipment, could transmission of electricity via
superconductivity be seriously considered.
In 1967,
Richard Garwin and Juri Matisoo at IBM published a paper
proposing the construction of a 100 GW, 1000 km, dc superconducting
transmission line based on the then newly discovered type II
compound, Nb
3Sn, refrigerated throughout its entire
length by liquid helium at 4.2 K. At the time it was thought remote
nuclear power plant farms or hydroelectric facilities would provide
a major portion of the then burgeoning national demand for
electricity, and that the “high power bandwidth” transmission at
near zero loss available from deployment of superconducting cables
would become economical. In principle, their idea presaged many
aspects of the SuperGrid concept. In the 1970s and early 1980s,
more studies on the feasibility of both ac and dc superconducting
cables appeared, and two
watershed ac
superconducting cables were built and successfully tested at
Brookhaven, NY, and Graz, Austria, the latter actually undergoing
live grid service for several years. At least two reports published
during this period explored the joint use of hydrogen with
superconducting wires for electricity transmission.
Bartlit, Edeskuty and Hammel considered an energy transmission
line employing low temperature superconductors cooling by liquid
helium with liquid hydrogen serving as a heat shield, the hydrogen
to be delivered eventually as rocket fuel for NASA. In 1975, a
report assembled by
Stanford University and NIST examined the use of “slush
hydrogen” at 14 K as cryogen for a cable using Nb3Ge with
a transition temperature near 20 K as the superconductor; however,
no attention was given the use of hydrogen as an energy agent
itself.
Following on the discovery of high
temperature superconductors in 1986 and the appearance of practical
tape and wire in the early 1990s,
Schoenung, Hassenzahl
and Grant revisited the work of
Garwin and Matisoo in light of these new events, and concluded
that an HTSC dc “electricity pipeline” cooled by liquid nitrogen
could compete economically with conventional high voltage dc
transmission lines or gas pipelines for distances greater than 200
km. Although today several prototype
HTSC superconducting cable demonstrations are planned or
actually undergoing test worldwide, all target ac applications at
transmission and distribution voltage levels at 66 kV and greater,
we must emphasize that the major advantage of superconductivity is
the ability to transport very large dc currents at relatively low
voltage. Only under constant current conditions are superconductors
perfect conductors, otherwise heat-producing hysteretic losses occur
requiring additional cryogenic capacity above and beyond that to
remove ambient thermal in-leak to the cable. Moreover, the use of
lower voltages will reduce dielectric stress and improve cable
reliability and extend lifetime.
Although the elements of a
SuperCable, delivering both electrical and chemical power, are
conceptually simple, for capacities on the scale of 5 GWe via
superconducting wire and 10 GWth via chemical potential energy in
the form of hydrogen or methane for a distance on the order 1000 km,
it’s actual construction would be an immense undertaking, rivaling
today’s natural gas pipeline expansion. The provision of
refrigeration and vacuum infrastructure along the right-of-way alone
would require deployment of these technologies on an unprecedented
level, surpassing even those servicing the largest hadron colliders
in existence or under plan.
Some idea of these requirements can be
culled from the seminal paper by Garwin and Matisoo (
GM)
published in 1967 proposing a 1000 km, 100 GW superconducting dc
cable, and the re-visitation of the concept in 1997 by Schoenung,
Hassenzahl and Grant (
SHG) in the
context of high temperature superconducting materials not available
to the former. Useful background is also provided by the late-90s
study at Fermilab of an 233-km circumference low-field
100 TeV hadron collider
energized by a low temperature superconducting carrying 100,000 A
dc.
From a macroscopic standpoint, the
construction of a SuperCable with these kinds of
specifications bears great similarity to that of several high
capacity, long distance natural gas pipelines under consideration
today, such as the 1300-km, 30-in, 18 GW
th
Mackenzie
Valley Project to run from gathering well fields near the
Mackenzie River – Artic Ocean Delta southward to the northern border
of Alberta.
In fact, this pipeline provides a useful
exercise for examining the effort and resource the Mackenzie Project
will require as a scoping scenario for a SuperCable.
-
To cost $3 B USD
-
Construction to
begin 2006 after seven years of environmental studies, 20 years
of negotiation with native population, five years
pre-permitting.
-
Commissioning and
delivery of up to 1.2 B cu-ft/day in 2009 – 2010 to northern
Alberta with fan-out to Eastern Canada and Mid-West US on
existing pipeline network.
-
Will employ up to
7000 workers housed in 40 “temporary villages.”
-
Will consume 5
cubic football fields of sand and gravel (granular
particulates).
-
Principal
transportation of material, heavy construction equipment and
pump station machinery will be over specially built barges to
perhaps ten landings to be constructed along the banks of the
Mackenzie River.
-
Construction of
the pipeline proper to take place over three winter seasons with
transportation over “artic roads” to be rebuilt every year.
Pipe will be laid in backfilled trenches. Some helicopter
delivery is anticipated.
-
Four or five
re-pressuring stations will be positioned along the route.
-
The width of the
right-of-way will be 40 - 50 meters, with pipe laid in surface
trenches 2 - 3 meters deep, except for "bridges" across
intervening rivers and streams.
-
Some
“manufacturing activity” (welding and lining of pipe) will be
done on-site.
Similar “macroscopic” issues are to be
expected with respect to the “laying” of a SuperCable, especially
with respect to right-of-way if trenching and surface construction
techniques are employed.
On the other hand, whole new issues arise
should "deep tunneling" be considered a possible venue for the
SuperCable. What differentiates tunneling for the SuperCable
from tunnels that supply water to New York or highways under the
Alps is distance...a few kilometers vs. hundreds. I know of only
one carefully thought out study of truly long distance underground
tunnels -- that mentioned above concerning a proposed future
low-field
hadron collider
located at Fermilab to be housed in a 233-km, 4-m diameter, long
circumferential tunnel, 50-m below the surface. The tunnel
would be excavated and mud removed by a series of novel,
battery-powered tunnel
boring machines. A lot of work has been undertaken at
Fermilab on the issues -- mechanical, geological and legal --
surrounding deep tunnel boring that could apply to the SuperCable.
Due to the special requirements to
support superconductivity, there are a number of “microscopic”
aspects present for the SuperCable that are absent in gas pipeline
construction. These “peculiarities” will heavily impact local
construction methods to be employed in building the SuperGrid.
Drawing on the GM and SHG studies, and
experience being gained at present designing, manufacturing and
deploying
prototype high temperature
superconducting (HTS) cables, the following should be noted:
Present HTS wire
technology is capable of manufacturing high performance tapes of
2-3 kilometers in length in production volumes exceeding 100,000
kilometers per year (there are only two such facilities
worldwide, however). There is no length limitation that does
not also practically govern aluminum or copper wire. On the
other hand, the current “1G” wire is 65% silver by volume and
thus expensive.
Although the HTS
wires for cables operate quite satisfactorily at the relatively
“hot” temperature of boiling liquid nitrogen, extensive
refrigeration an vacuum pumping equipment is required in
support. The line-length heat sources are, in order of
severity, for a dc cable:
-
Radiative heat
in-leak, 300 – 77 K
-
Turbulent flow
friction heat from flowing cryogens and/or gases
-
Type II
hysteretic losses due to current ripple superposed on the dc
level
-
Convection
losses through the cryostat vacuum, 300 – 77 K, which must
be maintained at 10-5 torr or lower throughout
the length of the cable.
-
Thermal
conductivity through internal support infrastructure (e.g.,
struts and spacers between concentric tubular structures.
The SHG report
estimated for a 1650 km line, vacuum stations would be needed
every kilometer and re-cooling refrigerators every 10. Each of
these units requires electric power. How is this to be
supplied?. An ancillary conventional power line in parallel with
the SuperCable? Tap offs from the superconducting cable with
inverters? Tap off of hydrogen to power a fuel cell supply? Or
some combination of all?
How should the
immense energy stored in the magnetic field surrounding the
100,000 amp cable conductor in the event of a fault be
“dumped?” GM suggest a parallel resistive shunt that would
dissipate that energy to the environment and help damp the
resulting L (di/dt) high voltage transients. Perhaps this could
be the same line as that supplying power to vacuum pumps and
refrigeration compressors.
All these “micro-issues” complicate
construction of the SuperCable significantly beyond that experienced
with gas pipelines. Having said that, there are several common
challenges. Among these are:
Size of the
“structure quanta” to be transported to the construction site,
e. g., length of each pipe (or SuperCable) segment to be
transported to the construction site. This is essentially
limited by the length of a truck trailer or railroad car
(crudely the distance between home plate and pitcher's mound,
roughly 20 meters, but could be much longer by barge (how do you
get the stock to the barge landing?).
-
Sidebar.
During the westward expansion of the railroad, rail stock
and laborer quarters and supplies simply followed, as the
track was extended…an option not available to us.
-
‘Coptering is
a possibility. I have seen ski lift towers put in place in
a matter of days once footings were laid and the structures
pre-fabed in the area parking lot.
If the SuperCable
“quanta” consisted of a prefabricated flexible tubular
structure that could be coiled and spooled, in principle
transportation of larger sections could be accomplished. Nexans
has estimated the
maximum length of a flexible superconducting cable of
nominal overall diameter 20-cm that could be built and spoiled
for “conventional” shipment by truck or barge is 600 meters.
Given the possibly more complex design of the SuperCable, and
especially the “hybrid-SuperCable” involving LN2, pressurized
supercritical H
2 and a superconducting conductor
(utility parlance has it that a “conductor” is something you
wind with wires, and a cable is what you put around the
“conductor.” A “conductor” becomes a “line” if you’re talking
about overhead transmission…confusing to the layman…nonetheless
we will try to stick to this usage herein).
These considerations give rise to
additional “on-site” construction challenges unique to the
SuperCable.
Creation and
maintenance of sufficient vacuum in the cryostat.
-
All present
and planned demonstrations of HTS ac cables, save one,
intend to continuously pump on the cryostat portion of the
cable assembly during operation. The sustaining of this
vacuum proved to be the Achilles' Heel of the
Pirelli-Detroit Edison Frisbie Substation demonstration.
Pirelli had employed welding and final inspection techniques
appropriate to hermetically sealed conventional underground
cables (this is my impression from what was actually
fabricated, not directly substantiated by Pirelli). This
weld failed on installation.
-
On the other
hand, the LIPA cable
installation being undertaken by AMSC and Nexans will use
permanently evacuated, to !0-6 torr, 100 meter
segments separated from each other. Nexans has been quite
successful providing cryogenic transfer lines of total
length several hundred meters to the European Space Program,
with vacuum integrity lifetime guaranteed.
On-site
fabrication of the “conductor.” First of all, the manufactured
wire length is currently limited to about three kilometers.
Although this could be extended to perhaps five. Depending on
the “pitch angle” the tape is wound on the conductor, its total
length could be a kilometer or more. This is in far excess of
the practical length that a rigid or flexible SuperCable
“package” could be fabricated in the factory and subsequently
shipped. However, a much longer flexible conductor,
perhaps 2 - 3 kilometers, of small diameter (2 - 3 cm) could be
"plant fabricated" then coiled, shipped and threaded through a
pre-laid rigid housing. This would be straightforward for
a monopole design, not so simple for coaxial.
Joining and
jointing. This is far more complicated task, for reasons listed
above, for the SuperCable than for gas pipelines, which involves
more than “simple welding and coating” they require. The
complication is exacerbated if the optimal design is shown to be
coaxial instead of two independent monopoles.
-
The
manufactured wire length is currently limited to about three
kilometers. Although this could be extended to perhaps
five. Depending on the “pitch angle” the tape is wound on
the conductor, an electrical joint or splice will be
required every kilometer.
-
A fair amount
of experience on the design and construction of HTS
superconducting joints exists already by virtue of past and
present cable prototype efforts in the US, Europe and Japan.
However, these joints are not "perfectly conducting" as they
are in MRI magnets. A specification issue for the
SuperCable would be the relative level of hysteretic losses
due to ripple compared to resistance in the joints.
The joint locations may also provide an opportunity for the
installation of fault current shunts of the type discussed
earlier.
Taking all the above under consideration,
we propose several rough monopolar SuperCable designs along the
following lines:
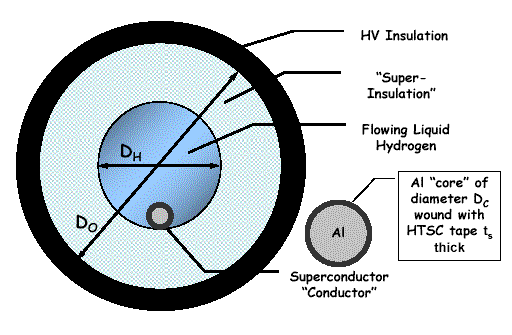
Essentially, this is the configuration of
a "gas pipe," except that it carries liquid hydrogen with a flexible
superconducting "conductor," overall diameter, D
C, with
superconducting tapes or wires of thickness tS laid
around a stranded aluminum cable, laying along the bottom of the
hydrogen transport tube of diameter, DH, and cooled by
flowing hydrogen slightly sub-cooled to 20 K, held under 2 - 7
atmospheres of pressure. The overall diameter, D
O,
approximately 2 × DH, is set by the layers of
super-insulation and thickness of the high voltage insulation
depicted by the outer black ring. All surfaces out to D
O
are "at potential," and reflect the "room temperature dielectric"
nature of the monopole approach, allowing normal dielectric
materials to be employed.
This design is actually quite simple.
One can envision concentric 20-m sections of the outer pipe,
enclosing D
H and DO, containing both the
electrical and thermal insulation, factory prefabricated and
shipped to the site and joined in 5-piece sections (100-m), and
then evacuated to 10
-5 torr and "permanently" sealed
with annular "walls" at each end. Subsequently, five of
these completed 100-m segments are connected to form a kilometer
unit. At this point, a 1000-m continuously long flexible
superconducting "conductor" is unreeled from its spool and
threaded and laid as shown in the above figure and jointed to
the next conductor, threaded through another 1000-m
segment...and so on...and so on.
To put some practical dimensions on
this embodiment of the SuperCable, assume a total power flow of
15 GW...5 electrical ( 25,000 V bipolar at 100,000 A dc circuit)
and 10 "thermal" in the form of flowing liquid hydrogen.
These values approximate the Mackenzie Valley Pipeline, assuming
1/2 of the methane potential energy is converted to electricity
at wellheads in the Northwest Territory gathering fields. Taking
well-established values for HTS wire critical current and
reasonable levels of fluid flow velocities, we find the
following numbers for D
C and DH: